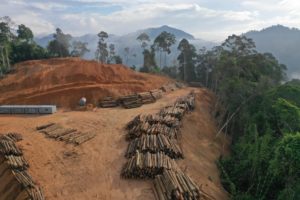
Photo above: Monocultural plantations of green palm oil in Indonesia. Adobe Photos
Written and edited by Hannah Evans, MA, Population Connection, and Janet Larsen, Principal, One Planet Strategies LLC
How Population Growth and Human Activities Increase the Risk of Zoonosis
The coronavirus pandemic highlights how the overuse of Earth’s natural systems poses a risk to human health and well-being.
While it is no surprise that the confluence of human population growth and expanding economies drives environmental degradation, scientists warn that failing to shrink humanity’s ecological footprint also increases the likelihood of new infectious disease outbreaks.
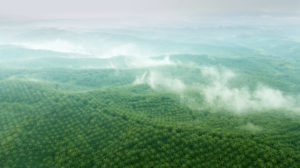
Avoiding future pandemics not only requires bolstering public health initiatives, but also reducing human impact on nature. This involves integrating a greater understanding of how human health and ecosystem health are interconnected and interact with social, political, and economic systems.
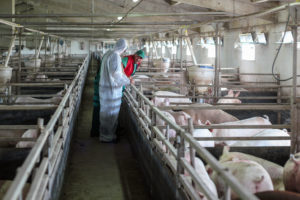
Population Growth and Zoonotic Diseases
The Covid-19 disease that emerged in China in late 2019 is caused by a novel coronavirus called severe acute respiratory syndrome coronavirus 2, or SARS-CoV-2. It is a zoonotic disease, meaning that it was transferred to humans from non-human animals.
Zoonotic diseases may be transmitted directly from contact with an infected animal or via vectors such as bacteria, parasites, or viruses in food, water, or soils [1]. In the case of Covid-19, the virus causing the disease was most likely harbored by bats [2]. Other well-known zoonoses include Ebola (also likely from bats), human immunodeficiency virus or HIV-1, the virus that causes AIDS (from chimpanzees), Zika virus (likely from monkeys), and avian and swine influenza (from poultry and pigs, respectively) [2-6]. Older human diseases, such as measles and smallpox, likely have an animal origin as well [7, 8].
The World Health Organization (WHO) notes that more than 200 diseases or infections have jumped to humans from other animals [9]. An estimated 75% of newly emerging infectious diseases are zoonotic [10]. Far more viruses in the animal kingdom have the potential to infect humans; scientists estimate that nearly 1.7 million undiscovered viruses exist in mammals and birds alone [11].
Infectious disease emergence is closely linked to global population growth. Animal-to-human viral spillovers are increasing because the growing human population is encroaching on animal habitats and disrupting ecosystems [12]. Adding more people to a finite planet increases the likelihood of animal-human interaction. This risk has been exacerbated by the rapid and continued growth of the human population over the past two centuries. For example, it took thousands of years for human numbers to reach 1 billion and just 200 more to reach 7 billion (see Figure 1) [13]. Although growth is slowing, human ranks are projected to expand into the next century. The United Nations’ medium variant projection shows the global population increasing to 8.5 billion in 2030 and 9.7 billion in 2050, eventually reaching 10.9 billion by the year 2100 [14].
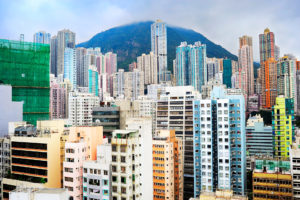
7.6 million residents with 68,400 people per square mile (2021). Adobe Photos
Researchers are continuously working to identify sites at risk of future zoonotic disease outbreaks. A 2008 study of diseases that emerged since 1940 found that densely populated areas with high levels of biodiversity that are experiencing rapid environmental changes are likely hotspots [15] (see Box on Biodiversity and Disease). While people in densely populated urban areas generally have less interaction with wildlife than people in rural areas or on the urban-rural frontier, once a disease takes hold in a city, it can spread quickly [16]. In our highly mobile and interconnected world, travelers and migrants can quickly expand a disease’s geographical reach.
“Trafficking wild fauna and flora can significantly impact human health, national security and economic development” [17].
United Nations Office on Drugs and Crime (UNODC), 2020
Biodiversity and Disease
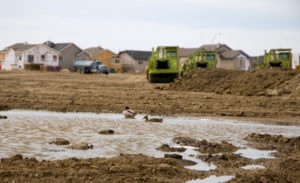
Within the context of zoonosis, the role of biological diversity is complex. The world’s most diverse ecosystems house a higher variety of organisms, including a wealth of pathogens that potentially could transfer to humans. However, biodiversity loss—often resulting from human disruption of habitat—can forge a clearer path for viruses to infect humans more directly.
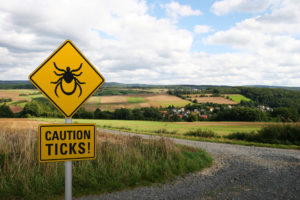
The species likely to survive habitat loss and fragmentation tend to be animals that are common hosts of diseases that can infect humans [18]. In a global survey, ecologists found that rodent, bat, and bird species known to host pathogens and parasites are more prevalent in human-dominated landscapes than in undisturbed areas [19, 20]. For example, research has shown that the human risk of contracting both West Nile virus and Lyme disease is much higher in human-dominated landscapes with low levels of biodiversity—namely suburbs and urbanized areas—in which a small number of hearty animal types have displaced the preexisting variety of native vertebrate populations [21]. Conversely, high levels of biodiversity and a higher prevalence of native vertebrates—as found in large intact forests—reduce human risk because mosquito and tick vectors can feed from a larger pool of host animals, most of which are unlikely reservoirs for pathogens. This results in lower infection rates in humans.
Human Activities Increase the Risk of Viral Spillover
Without changing the way people produce food and energy, population growth drives deforestation, habitat loss, and climate change. Together these processes amplify the threat of infectious disease outbreaks. From an epidemiological perspective, the human impacts most likely to put people at risk of contracting zoonoses include uncontrolled wildlife trade, intensified agriculture, habitat destruction, deforestation and other land use changes, antimicrobial resistance, and climate change [22].
Global Wildlife Trade
One important activity raising the risk of zoonotic disease outbreaks is wildlife exploitation. Trade in wildlife and wildlife products is a multibillion-dollar industry with complex supply chains, fueled in part by corruption [23-25]. Wild animals are harvested and sold for a number of reasons: as pets, as food, for medicine, and for luxury goods. Both wild and farmed species of exotic animals are traded, sometimes legally, other times outside the law. Exotic animals are more likely to harbor diseases that humans have not been exposed to and thus have no natural immunity to [26]. Illegal trade is particularly risky for human health because it evades health and safety controls. Moving protected species across international borders also violates the Convention on International Trade in Endangered Species of Wild Fauna and Flora (CITES), an international agreement signed by 183 governments that works to ensure that wildlife trade does not lead to species extinction [27].
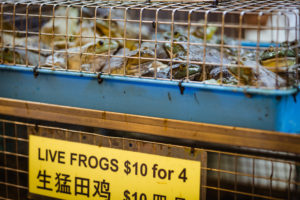
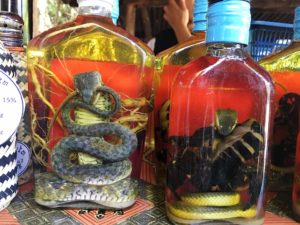
Wildlife trade has the potential to bring humans and domesticated animals into contact with novel pathogens throughout the supply chains—from the poachers and processors to the traders and consumers. Animals poached from their natural habitats may be sold locally, used as breeding stock, or exported, sometimes falsely labeled as captive-bred [28]. During transit and at markets, animals may be kept in crowded, unsanitary conditions and mixed with other exotic and domesticated animals—stresses and circumstances that can increase the likelihood of disease transfer among animals and across species [29]. Having vendors and customers in close proximity to a mix of wild animals and raw meat exacerbates the risk of disease spread [26].
The Covid-19 pandemic has incited countries around the world to work internally and along with organizations like the Global Program for Combating Wildlife and Forest Crime at the United Nations Office on Drugs and Crime to eliminate illegal wildlife trafficking. Failure to control and ultimately end the illegal wildlife trade could result in future disease outbreaks of pandemic potential [17]. Yet eliminating the trade and consumption of wildlife is difficult. Illegal wildlife traffic is elusive to track because illegal wildlife markets often exist alongside legal ones. Sales are aided by e-commerce and social media and abetted by insufficient or ineffective regulation and enforcement at local and national levels [30]. Demand for exotic live animals and meat is strong, for uses ranging from traditional medicine to luxury commodities. Meat from wild animals—including endangered or vulnerable species—is consumed for food, both as an accessible protein source among low-income populations as well as by wealthy people looking for an elite experience [31].
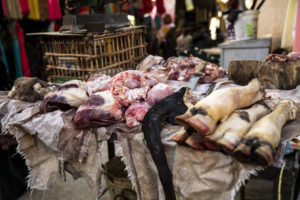
Strict bans on high-risk wildlife markets could push trade underground, making it harder to monitor [23]. Bans also jeopardize the livelihoods of millions around the world who depend on breeding and selling wild animals to survive. For example, the Chinese government’s ban on the consumption of wild animals following the Covid-19 outbreak undermines the $76 billion wildlife farming industry that the government had previously subsidized and the 15 million people it supports [32, 33]. Similar bans on the hunting, trade, and consumption of wild meat from animals, known colloquially as bushmeat, were implemented in response to Ebola outbreaks in West Africa in 2014 [34]. However, because bushmeat has historically served as a significant source of animal protein and income for people over centuries, the ban effectively multiplied informal wild animal trade networks while eroding public trust in health officials—in the words of one international research team, “rendering the development of acceptable, evidence-based surveillance and mitigation strategies for zoonotic spillovers almost impossible” [34]. Fortunately, the successful Ebola containment strategies implemented in and around Lagos, Nigeria, have invigorated hope among the global public health community (see Box on Ebola Virus).
Tackling the complex and globe-spanning problem of illegal wildlife trade requires an ongoing global effort and a greater understanding of the interconnected challenges underlying it, including the structure and incentives behind global supply chains, food supply issues, poverty, and corruption [35].
“Wild animals would not pass on these pathogens to humans if we didn’t bring them to our cities, markets, and shops. Illegally sourced wildlife traded in a clandestine way escapes any sanitary control and exposes human beings to the transmission of new viruses and other pathogens” [17].
UNODC, 2020
Disease in Focus: Ebola Virus
The Ebola virus is a deadly zoonotic disease that first emerged in 1976 in the Democratic Republic of the Congo (formerly Zaire). While the exact animal origin for the virus remains unknown, scientists believe that fruit bats serve as the disease reservoir, and that human contact with bats or nonhuman primates infected by bats initiated the pathogen transfer. In humans, the Ebola virus causes fevers and bleeding and spreads person-to-person via blood and other bodily fluids. Since its discovery, multiple Ebola outbreaks have occurred in sub-Saharan African countries, caused by several distinct viral strains [36].
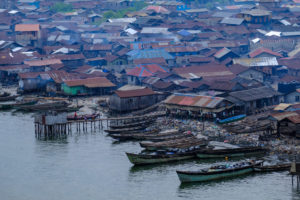
100 slums or informal settlements throughout the city. Adobe Photos
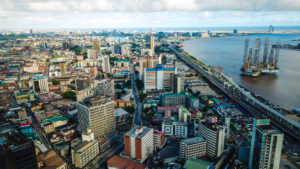
The largest Ebola outbreak to date occurred between the end of 2013 and 2016, centered in the West African countries of Guinea, Liberia, and Sierra Leone. It ultimately spread via travelers to Italy, Mali, Nigeria, Senegal, Spain, the United Kingdom, and the United States. More than 28,600 people were thought to have been infected, with 11,325 dying from the disease over this time period [37].
This 2013–2016 Ebola outbreak highlights the role that population dynamics play in the transmission of disease, in particular the rapid spread within crowded urban areas and across large distances as people routinely migrate from rural to urban areas and between countries and regions. In fact, West Africa’s population is estimated to be about seven times more mobile than populations elsewhere in the world [38].
These dynamics made the July 2014 discovery that Ebola had reached Lagos, Nigeria, a public health nightmare. Lagos is one of the fastest growing cities in the world and one of the most populous cities in all of Africa—the entire metro area was home to about 21 million people in 2014 [39]. The expansive city is also known for its crowded slums and extensive daily movement to and from the urban center. Interventions like contact tracing seemed futile in the face of high population density, high mobility, and wide disparities in wealth and stable infrastructure [38].
However, the Nigerian government worked quickly and in collaboration with international public health entities like WHO to contain the spread of the Ebola virus. For example, the city immediately imposed vigilant disinfection and port-of-entry screenings. An emergency operations center was created alongside the country’s existing virology laboratory to diagnose cases [38]. Importantly, potential contacts were traced, with more than 18,000 people visited and checked for fever and other symptoms [40]. Those showing symptoms were moved to isolation facilities [41]. The Nigerian government also launched educational campaigns to disseminate information and quell fears among the public. Using GPS data and mapping, Lagos reached 100% contact tracing [38].
Despite the very real demographic dangers Lagos faced, the government’s fast and collaborative response to the Ebola outbreak—made possible by the presence of an excellent public health infrastructure—helped avert what many assumed would be a deadly tragedy. WHO lauded the “spectacular success story” that limited Nigeria’s total caseload to 19 infections and seven deaths [38].
Intensified Agriculture
Another major factor increasing the risk of zoonotic disease outbreaks is the intensification of agriculture. Rather than spreading food production among many diversified farms cultivating a variety of plants and animals, industrial agricultural practices tend toward large, consolidated operations specializing in specific commodities, often reliant on inputs like chemical fertilizers, herbicides, and pesticides. Intensified agriculture commonly produces higher yields of fewer products on a given area of land. However, these systems also concentrate waste and chemical runoff, leading to air and water pollution, which poses health risks for people working and living in close proximity to these operations. Researchers link more than half of the communicable diseases newly transferred to humans from animals since 1940 to intensive agriculture [42].
One specific practice linked to disease spread is keeping large numbers of livestock in confined spaces, known as concentrated animal feeding operations, or CAFOs. While the United States relies most heavily on this approach to food production, producers in many other countries are following suit. The United Nations estimates that CAFOs supply 72% of the world’s poultry, 42% of eggs, and 55% of pork [43].
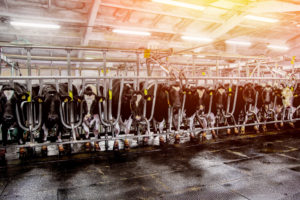
Intensive livestock farming facilitates disease transmission in several ways. Animals in CAFOs are often kept in crowded and stressful conditions and collectively produce large amounts of waste—ideal breeding grounds for infectious diseases. Waste runoff may enter waterways used by people and animals. Birds and other wild animals may make contact with waste held in pits or spread over fields. Air pollution is also a challenge: CAFO ventilation systems release material—including pathogens like the avian and swine influenza viruses—into the air, putting nearby wild and domesticated animals and humans in danger of being infected (see Box on Swine Flu) [22]. Flies, rats, and other vectors also spread pathogens [44]. Transmission works both ways, with pathogens moving from the wild to farmed animals and from farmed animals to the outside environment.
Other risky livestock-rearing practices include feeding diseased animals to other animals, which can make animals sick and transfer deadly pathogens to farmers and meat eaters. One such case that made international headlines as it disrupted beef supply chains and frightened beef consumers was “mad cow disease,” a fatal neurological disorder more formally called bovine spongiform encephalopathy [56]. BSE emerged in cows in the United Kingdom in the 1980s and, with its lengthy incubation period, showed up in people who had eaten infected beef a decade later as variant Creutzfeldt–Jakob disease [57]. The culprit was found to be meat and bone meal from infected cattle and sheep that was being fed to other cattle, animals that are biological herbivores.
CAFOs generally confine together large numbers of animals of the exact same breeds that share the same susceptibilities to diseases [45]. This low genetic diversity and high density can increase the probability of disease transmission and foster antibiotic resistance [58]. As a standard practice, animals in CAFOs are routinely dosed with antibiotics to prevent sickness and promote fast growth [22]. In fact, over 70% of antibiotics used worldwide go to animals raised for food [59]. The pre-emptive use of antibiotics contributes to the emergence of antimicrobial-resistant bacteria that cause infections—sometimes deadly ones—in both animals and humans, which cannot adequately be treated with existing antibiotics [60]. Such bacteria ends up on meat and other animal products that make their way to people via globalized food supply chains [61].
Disease in Focus: Swine Flu
Intensified agricultural practices that turn out large volumes of relatively inexpensive meat, milk, and eggs also make way for viruses to mutate to be transmitted to humans much more easily [44-46]. The 2009 H1N1 influenza pandemic—the first global flu pandemic in generations—illustrates how rapidly a disease can spread from industrial-scale food production facilities to people around the world [47]. The novel H1N1 influenza A virus likely originated in Mexican hog farms in 2009 and quickly spread to all 50 U.S. states and around the globe [48]. Ultimately, it infected people in more than 170 countries and killed up to 575,400 people within one year [49].
H1N1 viruses are common in pigs, which act as reservoirs for the disease. Researchers argue that the CAFO environment, which houses animals in crowded, indoor facilities by the thousands, is much more likely than smaller farms to propagate the evolution of such viruses [46]. CAFOs pose an even higher risk for prolonged spread of respiratory diseases because of the continual introduction of new animals into infected facilities. According to Dr. Gregory Gray, Director of the Center for Emerging Infectious Diseases at the University of Iowa College of Public Health, “When respiratory viruses get into these confinement facilities, they have continual opportunity to replicate, mutate, reassort, and recombine into novel strains” [46].
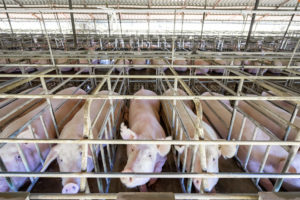
In fact, an earlier outbreak of a swine flu virus revealed variants containing proteins from swine flu, bird flu, and human flu viruses [50, 51]. This was first found in the United States in 1998 on a factory farm in North Carolina, a state where the population of hogs nearly equals that of humans [52, 53]. North Carolina hog farms are disproportionately located near low-income communities of color, furthering the environmental injustices associated with industrial agriculture operations [54]. With hog operations mostly located in areas with both high poverty and high percentages of nonwhites, the state’s most vulnerable populations also face an elevated risk of water contamination, air pollution, and viral infection. These populations suffer from especially high rates of certain health conditions potentially linked to CAFOs, such as anemia, kidney disease, tuberculosis, and septicemia, and have lower life expectancies than populations living farther from CAFOs [55]. The social, environmental, and economic dangers associated with such operations call into question their moral and ethical standing, especially as related to both environmental and public health injustices. Another major factor increasing the risk of zoonotic disease outbreaks is the intensification of agriculture. Rather than spreading food production among many diversified farms cultivating a variety of plants and animals, industrial agricultural practices tend toward large, consolidated operations specializing in specific commodities, often reliant on inputs like chemical fertilizers, herbicides, and pesticides. Intensified agriculture commonly produces higher yields of fewer products on a given area of land. However, these systems also concentrate waste and chemical runoff, leading to air and water pollution, which poses health risks for people working and living in close proximity to these operations. Researchers link more than half of the communicable diseases newly transferred to humans from animals since 1940 to intensive agriculture [42].
One specific practice linked to disease spread is keeping large numbers of livestock in confined spaces, known as concentrated animal feeding operations, or CAFOs. While the United States relies most heavily on this approach to food production, producers in many other countries are following suit. The United Nations estimates that CAFOs supply 72% of the world’s poultry, 42% of eggs, and 55% of pork [43].
Intensive livestock farming facilitates disease transmission in several ways. Animals in CAFOs are often kept in crowded and stressful conditions and collectively produce large amounts of waste—ideal breeding grounds for infectious diseases. Waste runoff may enter waterways used by people and animals. Birds and other wild animals may make contact with waste held in pits or spread over fields. Air pollution is also a challenge: CAFO ventilation systems release material—including pathogens like the avian and swine influenza viruses—into the air, putting nearby wild and domesticated animals and humans in danger of being infected (see Box on Swine Flu) [22]. Flies, rats, and other vectors also spread pathogens [44]. Transmission works both ways, with pathogens moving from the wild to farmed animals and from farmed animals to the outside environment.
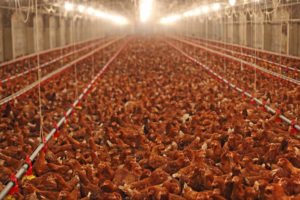
Other risky livestock-rearing practices include feeding diseased animals to other animals, which can make animals sick and transfer deadly pathogens to farmers and meat eaters. One such case that made international headlines as it disrupted beef supply chains and frightened beef consumers was “mad cow disease,” a fatal neurological disorder more formally called bovine spongiform encephalopathy [56]. BSE emerged in cows in the United Kingdom in the 1980s and, with its lengthy incubation period, showed up in people who had eaten infected beef a decade later as variant Creutzfeldt–Jakob disease [57]. The culprit was found to be meat and bone meal from infected cattle and sheep that was being fed to other cattle, animals that are biological herbivores.
CAFOs generally confine together large numbers of animals of the exact same breeds that share the same susceptibilities to diseases [45]. This low genetic diversity and high density can increase the probability of disease transmission and foster antibiotic resistance [58]. As a standard practice, animals in CAFOs are routinely dosed with antibiotics to prevent sickness and promote fast growth [22]. In fact, over 70% of antibiotics used worldwide go to animals raised for food [59]. The pre-emptive use of antibiotics contributes to the emergence of antimicrobial-resistant bacteria that cause infections—sometimes deadly ones—in both animals and humans, which cannot adequately be treated with existing antibiotics [60]. Such bacteria ends up on meat and other animal products that make their way to people via globalized food supply chains [61].
Habitat Destruction and Deforestation
Deforestation and land use change are major drivers of new infectious disease emergences in humans. The continuous growth of the human population has involved the progressive encroachment into natural frontiers and large-scale disruption of ecosystems and natural habitats. This brings humans into closer contact with wildlife and the diseases they harbor.
The correlation between deforestation and other natural habitat loss and disease emergence is well-established. A recent analysis of some 6,800 ecological communities on six continents adds to a growing body of research showing that land use changes are “creating expanding hazardous interfaces between people, livestock and wildlife reservoirs of zoonotic disease” [19]. This appears to be the case with Ebola outbreaks in Central and West Africa, for instance, which were associated with recent forest clearing [62].
Certain animals that harbor human-shared pathogens and parasites are more abundant in human-disturbed areas. As natural habitats become more fragmented by agricultural systems and human settlements, remaining wildland patches end up being too small to sustain large predators. As a result, small animals like rodents proliferate. For example, white-footed mouse populations—one of the vectors for the Lyme disease pathogen—thrive in North America’s fragmented forests. Studies have shown that the risk of Lyme disease infection in humans is much greater in smaller forest patches than in larger forests that sustain a more diverse ecosystem [16].
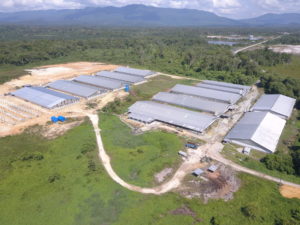
Malaria—caused by the potentially lethal Plasmodium parasite that is transferred to humans by mosquito bites—also has become increasingly prevalent with forest clearing [63]. Research in the Amazon rainforest has shown that cleared patches of forest and the partially shaded pools that form beside roads and in debris create ideal breeding habitat for disease-carrying mosquitoes [64, 65]. Irrigation channels also support mosquitoes as well as the snails that carry parasitic flatworms that cause schistosomiasis in humans [42]. Rapid deforestation and agricultural expansion have reversed the drop in malaria cases that followed the Brazilian government’s malarial control efforts in the 1940s and 1960s: while malaria cases in the Amazon Basin dropped precipitously from 6 million a year in the 1940s to 50,000 by the 1960s, they rebounded to over 600,000 by the year 2000 as more forest area was lost [66].
The Nipah virus is an example of a zoonotic disease that was transferred to humans because of environmental change and agricultural intensification. The virus, which can be asymptomatic or develop into an acute respiratory disease or brain inflammation in humans, was first recognized during an outbreak among pig farmers in Malaysia in 1998–1999 [67]. The virus has no cure and it kills an estimated 40 to 75% of people infected, dependent on local surveillance and health care [68].
Clearing rainforests for lumber, oil palm, and livestock production likely paved the way for the first Nipah outbreak. Scientists believe that fruit bats, displaced from their natural forest habitat, were attracted to the mango and other fruit trees surrounding pig farms. Pigs contracted Nipah by eating fallen fruit contaminated by bat feces and saliva, and then the virus jumped from the pigs to farmers and meat industry workers [69]. Since 2001, additional Nipah outbreaks have been recognized in Bangladesh and India, also associated with livestock infection and linked to contamination of food (most likely date palm sap) by fruit bats [67, 68]. Human-to-human transmission also occurred, via patient saliva. Public health experts warn that while Nipah outbreaks have thus far been containable, the potential exists for the disease to evolve to be more easily transmitted [70].
Interconnected Challenges
There are many complex and interconnected challenges facing our world today that contribute to the likelihood of disease transfer and future pandemics. In many ways, the very structure of the world economy that has developed since the Industrial Revolution endangers us, as it encourages large-scale production practices, specialized economies, and the overconsumption of fossil fuels that release greenhouse gases into the atmosphere, warming the climate (see Figure 2). Human activities like deforestation increase the risk of future pandemics. They also accelerate the trajectory of climate change, which in turn can act as a driver of infectious disease spread in a variety of ways, including by expanding or altering the geographical range of disease vectors like rodents and mosquitos [71].
For instance, as hotter temperatures allow mosquitoes to survive outside of their current habitats, the geographical range of diseases like malaria, dengue fever, chikungunya, and West Nile virus—and thus their human health toll—also expand [72]. Diseases once confined to the tropics could become commonplace in areas with more temperate climates, like much of the United States. The climate change-induced movement of species that host certain pathogens or parasites can trigger outbreaks in organisms that were previously unexposed.
A Way Forward: One Health and Nature-based Solutions
The Covid-19 pandemic has clarified the connections between human health and planetary health. A more symbiotic relationship between the environment and people is crucial to our survival; yet, human activities often undermine ecosystem health and the long-term ability of natural systems to support human well-being [73]. Major institutions such as WHO, the U.S. Centers for Disease Control and Prevention (CDC), and the United Nations are working to integrate these principles under the umbrella of One Health.
The One Health concept recognizes that the health of humans is intimately tied to the health of other living things. The goal is to improve human health outcomes by better appreciating the relationships between people, other animals, plants, and our shared environment. One Health works across disciplines at local, regional, national, and global levels [74]. The approach seeks to strengthen collaboration by enhancing communication, information sharing, and cooperation among various sectors including public health, medicine, scientific research, business, finance, agriculture, and labor. Integrating holistic and sustainable practices into the mainstream hinges on these vital connections.
Putting One Health concepts into practice is best approached situationally, but generally involves directly investing in integrated strategies that simultaneously address multiple societal and environmental problems [75]. These strategies include expanding scientific research into zoonotic disease emergence and surveillance and broader pandemic prevention approaches, as well as bolstering education about the connections between zoonosis and human activities. Furthermore, they involve adopting sustainable land management practices and developing alternatives for food security and livelihoods that do not rely on the destruction of habitats and biodiversity nor the exploitation of wild animals.
One of humanity’s most pressing challenges is how to feed a growing world population without further contributing to climate change and other forms of environmental degradation—both of which heighten the risk of future pathogen spillover. Globally, between 720 and 811 million people are undernourished [76]. With future food demand rising as population numbers and affluence grow, millions more people could suffer from food insecurity without improvements to global food production and distribution networks and a reduction in food waste [77]. Sustainable food production practices that minimize natural resource depletion, environmental degradation, and social inequalities are examples of Nature-based Solutions (NbS)—namely solutions that emphasize the roles that healthy natural systems play in addressing human challenges [78]. Through an NbS lens, ecosystem services, such as species and genetic diversity, freshwater purification, and soil vitality, are valued and integrated into food production systems.
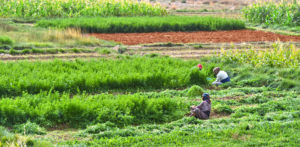
Nature-based Solutions in agriculture are myriad. For instance, they may involve integrating native flora into cattle pasture, restoring habitat for watershed health, or producing a variety of food and forest products on the same land [79]. An NbS approach could counter the lack of genetic diversity in modern food production systems and diets. According to the Food and Agriculture Organization of the United Nations, humans get over half of our food energy needs from just five crops: rice, wheat, maize, millet, and sorghum. Likewise, five animal species—cows, sheep, goats, pigs, and chickens—account for a third of average daily protein consumption [80]. A widespread plant disease or animal illness affecting any of these products could easily affect the diets of millions of people and farmer livelihoods. By design, NbS in agriculture favors mixed crop production and relies on fewer antimicrobial and synthetic fertilizers and chemical inputs than monocultural farming. This reduces the risk of pathogen spillover by mitigating antimicrobial resistance and biodiversity loss.
Furthermore, with many remedies for human ailments found in nature, taking care of natural systems preserves the possibility of finding more cures. While current short-term economic incentives favor high-production industrial agriculture, NbS takes a long-term view toward sustainability.
Final Thoughts
The stark reality we face is straightforward: When we destroy nature, we endanger ourselves. Zoonotic disease emergence is linked to human activities and exacerbated by population growth. The main processes that increase viral animal-to-human spillover are deforestation and habitat destruction, wildlife trade, industrial agriculture, and the chronic overuse of antibiotics. Greater human mobility, globalization, and international trade increase the chances that localized outbreaks reach pandemic scale. The future trajectory of each of these converging trends is partially dependent on the path that future fertility takes. Slowing population growth through voluntary measures, such as increasing access to family planning and education—especially for women and girls—has the capacity to improve the future of the planet and its inhabitants.
Access to sexual and reproductive health care can slow population growth through voluntary reductions in fertility. Quality comprehensive care enables women and couples to autonomously determine the number, timing, and spacing of their births. When reproductive autonomy is realized, many social, economic, and environmental benefits follow, including reductions in infant and maternal mortality increases in education rates for women and girls, improved livelihoods, poverty reduction, and reduced population pressures on natural resources, habitats, and food production systems.
The ability to control when, whether, and with whom to have a child is a fundamental human right. Yet there are 218 million women in low- and middle-income countries who want to avoid pregnancy but are not currently using any modern form of contraception [81]. In these regions alone, women have about 111 million unintended pregnancies annually. Research linking high fertility rates to high unmet need for family planning signals a need for increased investment in health and education.
Regardless of a country’s affluence, disparities in access to reproductive rights and quality health care exist throughout the world. While there is no available estimate of unmet need for family planning in the United States, for instance, research shows that 45% of pregnancies and over a third of births are unintended [82]. Especially given Americans’ high per capita consumption of fossil fuels and other natural resources, it is critical that the U.S. invest in voluntary family planning education and services both at home and overseas.
Reducing the risk of new disease emergence and the likelihood of creating the next pandemic depends on shrinking human impacts on other animals and the living environment. Addressing these intersecting global issues requires rethinking the economic drivers that fuel globalized trade, consumption patterns, waste generation, and environmental degradation. With agricultural expansion and intensification driving deforestation globally, future food production practices matter for disease spillover from nature.
Humanity’s environmental footprint is a function of human numbers and human consumption of resources. Integrating the interconnections between human health and ecosystem health into decisions and policies affecting land use, trade, development, and population growth becomes ever more important as human numbers grow and economies expand.
SOURCES
- Centers for Disease Control and Prevention (CDC). Zoonotic diseases. 2017; Available from: https://www.cdc.gov/onehealth/basics/zoonotic-diseases.html.
- Frutos, R., et al., COVID-19: Time to exonerate the pangolin from the transmission of SARS-CoV-2 to humans. Infection,
Genetics and Evolution, 2020. 84: p. 104493. - Carroll, D., et al., Building a global atlas of zoonotic viruses. Bulletin of the World Health Organization, 2018. 96(4): p. 292.
- Faria, N.R., et al., Zika virus in the Americas: Early epidemiological and genetic findings. Science, 2016. 352(6283): p. 345-349.
- Kock, R., et al., Searching for the source of Ebola: the elusive factors driving its spillover into humans during the West African outbreak of 2013–2016. OIE Scientific and Technical Review, 2019. 38(1): p. 113-117.
- Webster, R. and D. Hulse, Controlling avian flu at the source. Nature, 2005. 435(7041): p. 415-416.
- Morens, D.M., et al., Pandemic COVID-19 joins history’s pandemic legion. mBio, 2020. 11(3).
- Tournier, J.-N., Pandemic Legion History More Complex than Previously Thought. Mbio, 2020. 11(5).
- World Health Organization (WHO). Zoonoses. 2020; Available from: https://www.who.int/news-room/fact-sheets/detail/zoonoses.
- Gebreyes, W.A., et al., The global one health paradigm: Challenges and opportunities for tackling infectious diseases at the human, animal, and environment interface in low-resource settings. PLoS Neglected Tropical Diseases, 2014. 8(11): p. e3257.
- Carroll, D., et al., The global virome project. Science, 2018. 359(6378): p. 872-874.
- Vidal, J. Destroyed habitat creates the perfect conditions for coronavirus to emerge. Ensia, 2020.
- Roser, M., H. Ritchie, and E. Ortiz-Ospina, World population growth. Our World in Data, 2013.
- United Nations. World population prospects 2019: Highlights. 2019; Available from: https://population.un.org/wpp/Publications/Files/WPP2019_Highlights.pdf.
- Jones, K.E., et al., Global trends in emerging infectious diseases. Nature, 2008. 451(7181): p. 990-993.
- National Research Council, Sustaining global surveillance and response to emerging zoonotic diseases, G.T. Keusch, et al., Editors. 2009, National Academies Press: Washington, DC.
- UNODC, Preventing future pandemics of zoonotic origin by combating wildlife crime: Protecting global health, security and economy, in UNODC Guidance Note on COVID-19 links to Wildlife Crime. 2020, United Nations Office on Drugs and Crime: https://www.unodc.org/documents/Advocacy-Section/Wildlife_trafficking_COVID_19_GPWLFC_public.pdf.
- Tollefson, J., Why deforestation and extinctions make pandemics more likely. Nature, 2020. 584(7820): p. 175-176.
- Gibb, R., et al., Zoonotic host diversity increases in human-dominated ecosystems. Nature, 2020. 584(7821): p. 398-402.
- Ostfeld, R.S. and F. Keesing, Species that can make us ill thrive in human habitats. Nature 2020. 584: p. 346-347.
- Ostfeld, R.S., Biodiversity loss and the rise of zoonotic pathogens. Clinical Microbiology and Infection, 2009. 15: p. 40-43.
- Jones, B.A., et al., Zoonosis emergence linked to agricultural intensification and environmental change. Proceedings of the National Academy of Sciences, 2013. 110(21): p. 8399-8404.
- Targeting Natural Resource Corruption Project. Illegal wildlife markets, zoonotic disease transfer and corruption—
Connections and what the global community must do about it. TNRC Blog 2020; Available from: https://www.worldwildlife.org/pages/tnrc-blog-illegal-wildlife-markets-zoonotic-disease-transfer-and-corruption. - Outhwaite, W., Accessing, harvesting and trading in wildlife: Corruption in the use of permits and allocation of access rights,
in Targeting Natural Resource Corruption Topic Brief. 2020, TRAFFIC. - TRAFFIC. Illegal wildlife trade. 2020; Available from: https://www.traffic.org/about-us/illegal-wildlife-trade.
- Aguirre, A.A., et al., Illicit wildlife trade, wet markets, and COVID-19: Preventing future pandemics. World Medical & Health Policy, 2020. 12(3): p. 256-265.
- CITES. What is CITES? 2021; Available from: https://cites.org/eng/disc/what.php.
- Hall, J., Exotic pet trade, explained, in National Geographic. 2019.
- Baker, S.E., et al., Rough trade: animal welfare in the global wildlife trade. BioScience, 2013. 63(12): p. 928-938.
- Siriwat, P. and V. Nijman, Wildlife trade shifts from brick-and-mortar markets to virtual marketplaces: A case study of birds of prey trade in Thailand. Journal of Asia-Pacific Biodiversity, 2020. 13(3): p. 454-461.
- UNODC, World wildlife crime report 2020: Trafficking in protected species. 2020: United Nations Office on Drugs and Crime.
- Rammeloo, E. Coronavirus: China’s ban on wildlife trade hits animal breeders. Deutsche Welle July 16, 2020; Available from: https://www.dw.com/en/biodiversity-wet-markets-life-animals-covid-19-beijing-guangzhou/a-54183831.
- Arranz, A.H., Han. China’s wildlife trade. South China Morning Post March 4, 2020; Available from:
https://multimedia.scmp.com/infographics/news/china/article/3064927/wildlife-ban/index.html. - Bonwitt, J., et al., Unintended consequences of the ‘bushmeat ban’in West Africa during the 2013–2016 Ebola virus disease epidemic. Social Science & Medicine, 2018. 200: p. 166-173.
- de Wit, W., A. Freschi, and E. Trench, Covid 19: Urgent call to protect people and nature. 2020, WWF International: Gland,
Switzerland. - Centers for Disease Control and Prevention (CDC). Ebola (Ebola Virus Disease) website. 2020; Available from: https://www.cdc.gov/vhf/ebola/index.html.
- Centers for Disease Control and Prevention (CDC). 2014-2016 Ebola Outbreak in West Africa. 2019; Available from:
https://www.cdc.gov/vhf/ebola/history/2014-2016-outbreak/index.html. - World Health Organization (WHO). One year into the Ebola epidemic. 2015; Available from: https://www.who.int/news-room/spotlight/one-year-into-the-ebola-epidemic.
- Shuaib, F., et al., Ebola virus disease outbreak—Nigeria, July–September 2014. Morbidity and Mortality Weekly Report (MMWR), 2014. 63(39): p. 867-872.
- Courage, K.H., How did Nigeria quash its Ebola outbreak so quickly, in Scientific American. 2014.
- Fasina, F.O., et al., Transmission dynamics and control of Ebola virus disease outbreak in Nigeria, July to September 2014. Eurosurveillance, 2014. 19(40): p. 20920.
- Rohr, J.R., et al., Emerging human infectious diseases and the links to global food production. Nature Sustainability, 2019. 2(6): p. 445-456.
- Harvey, F., et al., Rise of mega farms: How the US model of intensive farming is invading the world, in The Guardian.
2017: https://www.theguardian.com/environment/2017/jul/18/rise-of-mega-farms-how-the-us-model-of-intensive-farming-is-invading-the-world. - Graham, J.P., et al., The animal-human interface and infectious disease in industrial food animal production: Rethinking biosecurity and biocontainment. Public Health Reports, 2008. 123(3): p. 282-299.
- Drew, T., The emergence and evolution of swine viral diseases: To what extent have husbandry systems and global trade contributed to their distribution and diversity? Revue Scientifique et Technique-OIE, 2011. 30(1): p. 95.
- Schmidt, C.W., Swine CAFOs & novel H1N1 flu: Separating facts from fears. Environmental Health Perspectives, 2009. 117(9):
p. A394-401. - Centers for Disease Control and Prevention (CDC). 2009 H1N1 pandemic timeline. 2019; Available from:
https://www.cdc.gov/flu/pandemic-resources/2009-pandemic-timeline.html. - Mena, I., et al., Origins of the 2009 H1N1 influenza pandemic in swine in Mexico. eLife, 2016. 5: p. e16777.
- Centers for Disease Control and Prevention (CDC). 2009 H1N1 pandemic (H1N1pdm09 virus). 2019; Available from:
https://www.cdc.gov/flu/pandemic-resources/2009-h1n1-pandemic.html. - MacKenzie, D., Swine flu: the predictable pandemic. New Scientist, 2009. 202(2706): p. 6-9.
- Zhou, N.N., et al., Genetic reassortment of avian, swine, and human influenza A viruses in American pigs. Journal of Virology, 1999. 73(10): p. 8851-8856.
- U.S. Census Bureau, U.S. and world population clock. 2020.
- U.S. Department of Agriculture, Quarterly hogs and pigs. 2020, National Agricultural Statistics Service (NASS),
Agricultural Statistics Board, USDA: Washington, DC. - Wing, S., D. Cole, and G. Grant, Environmental injustice in North Carolina’s hog industry. Environmental Health Perspectives,
2000. 108(3): p. 225-231. - Kravchenko, J., et al., Mortality and health outcomes in North Carolina communities located in close proximity to hog
concentrated animal feeding operations. North Carolina Medical Journal, 2018. 79(5): p. 278-288. - Centers for Disease Control and Prevention (CDC). Bovine Spongiform Encephalopathy (BSE), or Mad Cow Disease. 2018;
Available from: https://www.cdc.gov/prions/bse/about.html. - Lee, J., et al., Prion diseases as transmissible zoonotic diseases. Osong Public Health and Research Perspectives, 2013. 4(1):
p. 57-66. - Cha, W., et al., Comparing the genetic diversity and antimicrobial resistance profiles of Campylobacter jejuni recovered
from cattle and humans. Frontiers in Microbiology, 2017. 8: p. 818. - Van Boeckel, T.P., et al., Global trends in antimicrobial resistance in animals in low-and middle-income countries. Science, 2019. 365(6459).
- Gilchrist, M.J., et al., The potential role of concentrated animal feeding operations in infectious disease epidemics and antibiotic resistance. Environmental Health Perspectives, 2007. 115(2): p. 313-316.
- Van Boeckel, T.P., et al., Global trends in antimicrobial use in food animals. Proceedings of the National Academy of Sciences, 2015. 112(18): p. 5649-5654.
- Olivero, J., et al., Recent loss of closed forests is associated with Ebola virus disease outbreaks. Scientific Reports, 2017.
7(1): p. 1-9. - MacDonald, A.J. and E.A. Mordecai, Amazon deforestation drives malaria transmission, and malaria burden reduces forest
clearing. Proceedings of the National Academy of Sciences, 2019. 116(44): p. 22212-22218. - Chaves, L.S.M., et al., Abundance of impacted forest patches less than 5 km2 is a key driver of the incidence of malaria in
Amazonian Brazil. Scientific Reports, 2018. 8(7077): p. 1-11. - Vittor, A.Y., et al., Linking deforestation to malaria in the Amazon: Characterization of the breeding habitat of the principal
malaria vector, Anopheles darlingi. The American Journal of Tropical Medicine and Hygiene, 2009. 81(1): p. 5-12. - Zimmer, K., Deforestation is leading to more infectious diseases in humans, in National Geographic. 2019.
- Hughes, J.M., et al., Transmission of human infection with Nipah virus. Clinical Infectious Diseases, 2009. 49(11):
p. 1743-1748. - World Health Organization (WHO). Nipah virus. 2018; Available from: https://www.who.int/news-room/fact-sheets/detail/nipah-virus.
- Pulliam, J.R., et al., Agricultural intensification, priming for persistence and the emergence of Nipah virus: a lethal
bat-borne zoonosis. Journal of the Royal Society Interface, 2012. 9(66): p. 89-101. - Constable, H., The other virus that worries Asia, in BBC. 2021.
- Wu, X., et al., Impact of climate change on human infectious diseases: Empirical evidence and human adaptation.
Environment International, 2016. 86: p. 14-23. - Mordecai, E.A., et al., Detecting the impact of temperature on transmission of Zika, dengue, and chikungunya using
mechanistic models. PLoS Neglected Tropical Diseases, 2017. 11(4): p. e0005568. - Myers, S.S., et al., Human health impacts of ecosystem alteration. Proceedings of the National Academy of Sciences, 2013. 110(47): p. 18753-18760.
- Centers for Disease Control and Prevention (CDC). One Health basics. 2018; Available from:
https://www.cdc.gov/onehealth/basics/index.html. - Kelly, T.R., et al., Implementing One Health approaches to confront emerging and re-emerging zoonotic disease threats: Lessons from PREDICT. One Health Outlook, 2020. 2(1): p. 1-7.
- FAO, et al., The state of food security and nutrition in the world 2020. Transforming food systems for affordable healthy diets. 2020, U.N. Food and Agriculture Organization: Rome.
- Searchinger, T., et al., Creating a sustainable food future: A menu of solutions to feed nearly 10 billion people by 2050.
Final report. 2019, World Resources Institute, the World Bank Group, the United Nations Environment Programme, the United Nations Development Programme, the Centre de coopération internationale en recherche agronomique pour le développement, and the Institut national de la recherche agronomique: Washington, DC. - Cohen-Shacham, E., et al. (Eds.),, Nature-based solutions to address global societal challenges. 2016, IUCN: Gland,
Switzerland. p. 1-97. - Miralles-Wilhelm, F., Nature-based solutions in agriculture – Sustainable management and conservation of land, water,
and biodiversity. 2021, FAO and The Nature Conservancy: Virginia. - U.N. Food and Agriculture Organization, Biodiversity for sustainable agriculture: FAO’s work on biodiversity for food and
agriculture. 2019: http://www.fao.org/3/CA2227EN/ca2227en.pdf. - Sully, E., et al., Adding it up: Investing in sexual and reproductive health, 2019. 2020, Guttmacher Institute: New York.
- Guttmacher Institute. Unintended pregnancy in the United States. 2019; Available from: https://www.guttmacher.org/fact-sheet/unintended-pregnancy-united-states.